Real-time intraoperative ultrasound in brain surgery: neuronavigation and use of contrast-enhanced image fusion
Introduction
Point-of-care devices for intraoperative imaging represent one of the greatest adjuvant to modern strategies for surgical planning and execution; this is particularly true in neurosurgery given the complexity of the central nervous system and the delicate surgical maneuvers needed to manipulate it without causing iatrogenic damage. Preoperative imaging revolving around the correct prescription of specific MRI sequences (including digital tensor imaging and subsequent tractography analysis; arterial spin labeling; dynamic susceptibility contrast perfusion-weighted imaging and blood oxygenation level dependent, better known as BOLD sequences for functional MRI) allowed surgeons to obtain important information regarding the location of brain lesions and their relationship with surrounding eloquent anatomical structures (1-7). Together with data obtained through highly-selective nuclear medicine investigations, meant to disclose the metabolic behavior of those lesions, preoperative imaging serves as the first step in the planning of any neurosurgical intervention (8-11). To forecast the complexity of brain surgery and tackle it appropriately, most of those information can nowadays be tested through virtual surgery platforms; the final surgical plan is then implemented into the surgical theatre by making good use of surgical aids such as neuronavigation systems (see Figures 1,2), which can be easily integrated into operating microscopy (12-16). Nonetheless, all the efforts put into weighting the pros and cons of different approaches, and tailoring the treatment strategy around patient’s and lesion’s specific characteristics, cannot always prepare surgeons for unexpected intraoperative challenges, such as brain shift following cerebrospinal fluid (CSF) deliquoration, lack of well-defined microscopic boundaries, diffuse bleeding, etc. (17,18).
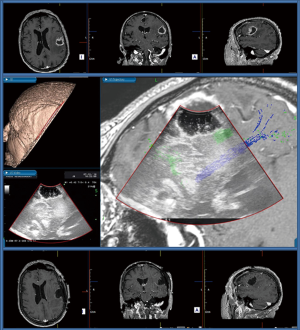
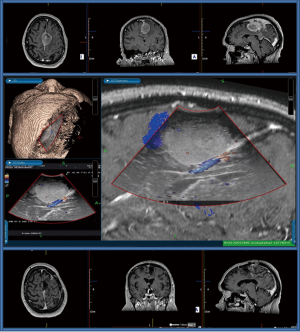
Hence the idea to leverage on point-of-care devices, such as 3D intraoperative ultrasound (IoUS), which can offer real-time imaging of any structure in the surgical field and help surgeons to correlate intraoperative anatomical images with those embedded preoperatively into neuronavigation systems (19). Since its inception in the last century, IoUS has initially evolved as a standalone option for real-time image guided surgery and is now becoming more interconnected with other technological aids meant to ensure greater safety and accuracy.
Here we will focus on the most relevant technical aspects behind the physics of IoUS and its application in brain surgery, aiming to define the wealth of opportunities, as well as the current challenges, of this powerful methodology.
Understanding the array of imaging modalities for IoUS
Following its introduction in neurosurgery in 1982 IoUS provided surgeons with the valuable opportunity to craft and update operative plans without using ionizing radiation, which had characterized the first decades of our specialty (20-22). IoUS systems exploit the so-called pulse-echo technique: in fact, they rely on piezoelectric transducers, emitting pulses at a frequency of 1–20 MHz, and on the recording of the echoed events produced by the sound pulses (23). Of note, depending on the peculiar acoustic properties of the tissues crossed, those pulses can either be absorbed, scattered, or reflected; this determines the different attenuation coefficient (expressed in dB/cm) of any given tissue (24,25). In a landmark laboratory study, conducted on autopsy human brain specimens, Kremkau et al. (26) found out that the average attenuation of the ultrasound pulse while crossing white and brain matter at a frequency of 1 MHz was 0.87 dB/cm, being higher in fiber tracts (0.97 dB/cm) than in isolated brain cortex (0.75 dB/cm). This and other seminal experimental contributions, demonstrated that the decay of the energy of the sound pulse is proportional to the distance from the emitting transducer: hence the amplitude of the ultrasound pulse progressively decreases as it propagates through the brain (24,26,27). As a result, we now know that the strength of the echoes is strictly related to the differences in acoustic properties of brain structures, and this determines the different degrees of brightness displayed in the monitor to the operator (28).
Preventing artifacts
Beside better understanding the physics of IoUS when it comes to investigate white and grey matters, neurosurgeons should also be well aware of the variety of possible artifacts that in not so experienced hands could lead to intraoperative misinterpretation. Selbekk et al. (23) investigated the most common reasons for imperfect ultrasound image, and concluded that they usually result as a violation of one or more of the fundamental assumptions for an ideal recording. Those assumptions can be summarized as follows:
- The ultrasound beam should be narrow with uniform width;
- The ultrasound waves should travel in a straight line directly to the reflecting object and back to the transducer;
- The speed of sound should be constant and known in any given soft tissue;
- The attenuation of ultrasound should be constant and uniform.
In fact, any limitation to the width of the beam, or the increase of noise proportionally to the depth of the surgical cavity, as well as the different attenuation coefficients of the intracranial structures, account for the violation of the above-mentioned assumptions. This said, it should be highlighted that the most common sources of errors are brightness artifacts in the surgical cavity caused by entrapped air bubbles, coagulated blood or advanced hemostatic agents (23,29,30). Furthermore, it is worth mentioning, as reported by Sastry et al. (31), that also the normal saline solution commonly used to fill the surgical cavity can produce an error of approximately 1.6 mm at a depth of 10 cm from the transducer, of note, they also added that even its temperature can potentially affect the quality of images obtained intraoperatively. As such to compensate for those artifacts, neurosurgeons should select the transducer most suitable to the size and shape of the craniotomy, as well as adapting the acquisition frequency to the location and sonographic properties of the lesion and its surrounding anatomy (23,32). Depending on the surgical scenario, it would be warranted to adopt one of the following strategies:
- A way to correct the attenuation of the sound pulse is called time-gain compensation: this strategy makes equally echogenic tissues look the same even if they are located at different depths (33-35).
- If possible the unscrubbed theatre team can help optimizing the orientation of the surgical table so that at time of IoUS the resection surface is kept horizontal with respect to gravity: this may help to minimize air trapping within the cavity (23,36).
- The operating surgeon should be aware of possible bone-induced high signal-attenuation and orientate the beam pulse accordingly (20,21,36).
- Whenever probes of small size and footprint are available, and the surgical cavity allows it, neurosurgeons can carefully attempt to explore its walls and inspect the resection margins: introducing the probe into the cavity can remarkably reduce, if not remove, the brightness artifacts and the mirroring/shadowing effects, since the distance between the transducer and possible residual foci becomes shorter (29,37).
- A qualitative stiffness assessment, conducted by strain sonoelastography, an ultrasound imaging technique able to assess mechanical properties of tissues should be considered to obtain a sharper visualization of the lesion margins compared to standard B-mode IoUS (32).
Accuracy of IoUS as an adjunct to neuronavigation
As any other non-frame-based navigation systems, also IoUS is subject to registration errors. The accuracy of IoUS was initially tested in laboratory setting and lately confirmed in surgical theatres. Lindseth et al. (19) described an accuracy of 1.40±0.45 mm (arithmetic mean) for ultrasound-based neuronavigation system, and highlighted that improper probe calibration was the major contributor to these accuracy errors. Of note, by simultaneously recording doppler and B-mode images, this accuracy can be further improved; Morin et al. (38) quantified this increase in over 10%, with a 67% correction of brain-shift. Similarly, by adopting Doppler IoUS images acquired on the surface of the dura to correct brain-shift and other sources of registration inaccuracies, Chen et al. (39) found that target registration errors (TRE) were less than 2.5 mm in more than 90% of cases. These figures were confirmed in clinical scenarios were the TREs was on average inferior than the 3 mm of standard neuronavigation based on preoperative MRI scans alone. Overall, these levels of accuracy are widely acceptable in standard clinical practice for excision of brain tumors as well as lesionectomies for functional neurosurgery, where the target is typically far larger than in stereotactic procedures (40). The reasons lie in the use of multiple other parameters that the operating surgeon takes into account to refine the indications provided by IoUS, and increase the extent of resection of brain lesions: including intraoperative neurophysiology, intraoperative fluorescent dyes, intraoperative Raman spectroscopy, nano-immunoassay-based microproteomic, etc. (1,2,12,41-51). This said, by fusing IoUS over preoperative MRI scans it is possible to definitely increase the overall accuracy of neuronavigation. This fusion is fully compatible with the surgical workflow in terms of execution times and user interactions (38). Additionally, by permitting multiple acquisitions, the frequent update of the image guidance system in all phases of the operation remarkably decrease if not completely remove inaccuracy issues (52,53).
Advantages and limitations of IoUS for brain surgery
Overall, IoUS has a significant edge over the other intraoperative aids for image guidance in brain surgery, especially in terms of independency, cost, and adaptability to multiple different clinical scenarios. By comparison with tools leveraging on preoperative neuroradiology and nuclear medicine investigations, IoUS is a compact and portable system that can independently provide real-time imaging. In fact, IoUS suites are not cumbersome and can be integrated into existing theatre infrastructure with minimal disruption to surgical workflow (29,38). The costs for acquisition and maintenance of IoUS suites are significantly cheaper when compared to any other intraoperative imaging tool for selective visualization of blood vessels or tumor residuals. Besides, IoUS comes across as a more flexible surgical companion since it serves many more purposes: identification of the CSF and blood flow, appreciation of the consistency, tissue density, peripheral infiltration or other physical properties of the lesions excised, and immediate visualization of complications, such as a hematomas. On the other hand, IoUS imaging is user dependent, with a steep learning curve, and specific metrics amenable for the characterization of brain lesions are warranted. Recently, Camp et al. (29) provided a significant contribution to this topic by describing new, reliable metrics (i.e., the Imperial tumor index, or the Infiltration index), eventually being able to correlate their IoUS findings with the histological grade of the lesions excised. The advances described above are nonetheless expected to be outpaced soon by new generations of IoUS suites with integrated algorithm for image analysis, and by the diffusion of contrast enhanced ultrasound imaging.
Contrast agents and the future of IoUS
The first and foremost goal of ultrasonic contrast agents is to enhance image-definition, offering the possibility to assess the blood flow within brain lesions through perfusion imaging of small capillary blood vessels (27,54). Many authors demonstrated that contrast enhanced IoUS combined with B-mode ultrasound improves tumor detection and resection control in neurosurgery, even in single ultrasound-guided operations (21,27,55,56). Systems for image fusion and virtual navigation combining intraoperative real-time IoUS with reconstructive preoperative coplanar MRI are already available for image-guided resection of high-grade gliomas. Those systems are nowadays more user-friendly and suitable even for neurosurgeons who lack the expertise in ultrasound technology. Wu et al. (57) reported that image fusion of contrast enhanced IoUS allows to discern the brain structure and obtain a better recognition, in real time and in multiplane from different angles, of the tumor/edema interface compared with reconstructive preoperative coplanar-enhanced MRI. Noteworthy, microbubbles and nanoparticles contrast agents have been recently proposed as the latest innovation for ultrasound imaging: following endovenous administration they are free to circulate in the blood stream and because of their micrometer or nanometer size and the binding ligands on their surfaces, can bypass vascular endothelial cells and accumulate at tissue sites that over-express those molecular targets (54,58-60).
Conclusions
IoUS has certainly all that it takes to be considered as an excellent tool for surgical planning and real-time image-guided excision of intracranial lesions. Neurosurgical/Anatomical courses on the use of IoUS are becoming more common and will likely increase in the coming decade, when further technical refinement will enhance image definition and expand the use of this methodology. The importance of the diffusion of those courses is striking, because the past generation of neurosurgeons had to rely solely on intraoperative practice to improve their mastery of brain ultrasound (61). Going forward, it is easy to forecast that official diplomas and certification will be offered by national and international neurosurgical societies to ensure homogeneous teaching standards, skills requirements and revalidation practices.
Acknowledgements
None.
Footnote
Conflicts of Interest: The authors have no conflicts of interest to declare.
References
- Talacchi A, Turazzi S, Locatelli F, Sala F, Beltramello A, Alessandrini F, Manganotti P, Lanteri P, Gambin R, Ganau M, Tramontano V, Santini B, Gerosa M. Surgical treatment of high-grade gliomas in motor areas. The impact of different supportive technologies: a 171-patient series. J Neurooncol 2010;100:417-26. [Crossref] [PubMed]
- Ganau L, Paris M, Ligarotti GK, Ganau M. Management of Gliomas: Overview of the Latest Technological Advancements and Related Behavioral Drawbacks. Behav Neurol 2015;2015:862634. [Crossref] [PubMed]
- Zeng Q, Jiang B, Shi F, Ling C, Dong F, Zhang J. 3D Pseudocontinuous Arterial Spin-Labeling MR Imaging in the Preoperative Evaluation of Gliomas. AJNR Am J Neuroradiol 2017;38:1876-83. [Crossref] [PubMed]
- Ma H, Wang Z, Xu K, Shao Z, Yang C, Xu P, Liu X, Hu C, Lu X, Rong Y. Three-dimensional arterial spin labeling imaging and dynamic susceptibility contrast perfusion-weighted imaging value in diagnosing glioma grade prior to surgery. Exp Ther Med 2017;13:2691-8. [Crossref] [PubMed]
- Lindner T, Ahmeti H, Lübbing I, Helle M, Jansen O, Synowitz M, Ulmer S. Intraoperative resection control using arterial spin labeling - Proof of concept, reproducibility of data and initial results. Neuroimage Clin 2017;15:136-42. [Crossref] [PubMed]
- Zacà D, Jovicich J, Nadar SR, Voyvodic JT, Pillai JJ. Cerebrovascular reactivity mapping in patients with low grade gliomas undergoing presurgical sensorimotor mapping with BOLD fMRI. J Magn Reson Imaging 2014;40:383-90. [Crossref] [PubMed]
- Gulati S, Berntsen EM, Solheim O, Kvistad KA, Håberg A, Selbekk T, Torp SH, Unsgaard G. Surgical resection of high-grade gliomas in eloquent regions guided by blood oxygenation level dependent functional magnetic resonance imaging, diffusion tensor tractography, and intraoperative navigated 3D ultrasound. Minim Invasive Neurosurg 2009;52:17-24. [Crossref] [PubMed]
- Braun V, Dempf S, Tomczak R, Wunderlich A, Weller R, Richter HP. Multimodal cranial neuronavigation: direct integration of functional magnetic resonance imaging and positron emission tomography data: technical note. Neurosurgery 2001;48:1178-81. [PubMed]
- Ganau M, Syrmos N, Ligarotti GK, Ganau L, Prisco L. Postoperative granulomas versus tumor recurrence: PET and SPET scans as strategic adjuvant tools to conventional neuroradiology. Hell J Nucl Med 2012;15:184-7. [PubMed]
- Stockhammer F, Misch M, Horn P, Koch A, Fonyuy N, Plotkin M. Association of F18-fluoro-ethyl-tyrosin uptake and 5-aminolevulinic acid-induced fluorescence in gliomas. Acta Neurochir (Wien) 2009;151:1377-83. [Crossref] [PubMed]
- Tanaka Y, Nariai T, Momose T, Aoyagi M, Maehara T, Tomori T, Yoshino Y, Nagaoka T, Ishiwata K, Ishii K, Ohno K. Glioma surgery using a multimodal navigation system with integrated metabolic images. J Neurosurg 2009;110:163-72. [Crossref] [PubMed]
- Ganau L, Ligarotti GKI, Ganau M. Predicting complexity of tumor removal and postoperative outcome in patients with high-grade gliomas. Neurosurg Rev 2018;41:371-3. [Crossref] [PubMed]
- Sawaya R, Alsideiri G, Bugdadi A, Winkler-Schwartz A, Azarnoush H, Bajunaid K, Sabbagh AJ, Del Maestro R. Development of a performance model for virtual reality tumor resections. J Neurosurg 2018;1:1-9. [Crossref] [PubMed]
- Sboarina A, Foroni RI, Minicozzi A, Antiga L, Lupidi F, Longhi M, Ganau M, Nicolato A, Ricciardi GK, Fenzi A, Gerosa M, De Simone A, Fracastoro G, Guglielmi A, Cordiano C. Software for hepatic vessel classification: feasibility study for virtual surgery. Int J Comput Assist Radiol Surg 2010;5:39-48. [Crossref] [PubMed]
- Dakson A, Hong M, Clarke DB. Virtual Reality Surgical Simulation: Implications for Resection of Intracranial Gliomas. Prog Neurol Surg 2018;30:106-16. [PubMed]
- Wang D, Ma D, Wong ML, Wáng YX. Recent advances in surgical planning & navigation for tumor biopsy and resection. Quant Imaging Med Surg 2015;5:640-8. [PubMed]
- Iversen DH, Wein W, Lindseth F, Unsgård G, Reinertsen I. Automatic Intraoperative Correction of Brain Shift for Accurate Neuronavigation. World Neurosurg 2018;120:e1071-8. [Crossref] [PubMed]
- Gerard IJ, Kersten-Oertel M, Petrecca K, Sirhan D, Hall JA, Collins DL. Brain shift in neuronavigation of brain tumors: A review. Med Image Anal 2017;35:403-20. [Crossref] [PubMed]
- Lindseth F, Langø T, Bang J. Nagelhus, Hernes TA. Accuracy evaluation of a 3D ultrasound-based neuronavigation system. Comput Aided Surg 2002;7:197-222. [Crossref] [PubMed]
- Unsgaard G, Rygh OM, Selbekk T, Müller TB, Kolstad F, Lindseth F, Hernes TA. Intra-operative 3D ultrasound in neurosurgery. Acta Neurochir (Wien) 2006;148:235-53. [Crossref] [PubMed]
- Unsgaard G, Ommedal S, Muller T, Gronningsaeter A, Nagelhus Hernes TA. Neuronavigation by intraoperative three-dimensional ultrasound: initial experience during brain tumor resection. Neurosurgery 2002;50:804-12. [Crossref] [PubMed]
- Ganau M, Syrmos N, Martin AR, Jiang F, Fehlings MG. Intraoperative ultrasound in spine surgery: history, current applications, future developments. Quant Imaging Med Surg 2018;8:261-7. [Crossref] [PubMed]
- Selbekk T, Jakola AS, Solheim O, Johansen TF, Lindseth F, Reinertsen I, Unsgård G. Ultrasound imaging in neurosurgery: approaches to minimize surgically induced image artefacts for improved resection control. Acta Neurochir (Wien) 2013;155:973-80. [Crossref] [PubMed]
- Strowitzki M, Brand S, Jenderka KV. Ultrasonic radio-frequency spectrum analysis of normal brain tissue. Ultrasound Med Biol 2007;33:522-9. [Crossref] [PubMed]
- Pichardo S, Sin VW, Hynynen K. Multi-frequency characterization of the speed of sound and attenuation coefficient for longitudinal transmission of freshly excised human skulls. Phys Med Biol 2011;56:219-50. [Crossref] [PubMed]
- Kremkau FW, Barnes RW, McGraw CP. Ultrasonic attenuation and propagation speed in normal human brain. J Acoust Soc Am 1981;70:29-38. [Crossref]
- Yan F, Song Z, Du M, Klibanov AL. Ultrasound molecular imaging for differentiation of benign and malignant tumors in patients. Quant Imaging Med Surg 2018;8:1078-83. [Crossref] [PubMed]
- Xiao J, Xiao M, Wang B, Huang Z, Peng K. Acoustic field simulation method for arbitrarily shaped transducer with dynamically refined sub-elements. Quant Imaging Med Surg 2018;8:1084-94. [Crossref] [PubMed]
- Camp SJ, Apostolopoulos V, Raptopoulos V, Mehta A, O'Neill K, Awad M, Vaqas B, Peterson D, Roncaroli F, Nandi D. Objective image analysis of real-time three-dimensional intraoperative ultrasound for intrinsic brain tumour surgery. J Ther Ultrasound 2017;5:2. [Crossref] [PubMed]
- Georgiadis D, Uhlmann F, Lindner A, Zierz S. Differentiation between true microembolic signals and artefacts using an arbitrary sample volume. Ultrasound Med Biol 2000;26:493-6. [Crossref] [PubMed]
- Sastry R, Bi WL, Pieper S, Frisken S, Kapur T, Wells W 3rd, Golby AJ. Applications of Ultrasound in the Resection of Brain Tumors. J Neuroimaging 2017;27:5-15. [Crossref] [PubMed]
- Prada F, Del Bene M, Rampini A, Mattei L, Casali C, Vetrano IG, Gennari AG, Sdao S, Saini M, Sconfienza LM, DiMeco F. Intraoperative Strain Elastosonography in Brain Tumor Surgery. Oper Neurosurg (Hagerstown) 2018. Epub ahead of print. [Crossref] [PubMed]
- Pye SD, Wild SR, McDicken WN. Adaptive time gain compensation for ultrasonic imaging. Ultrasound Med Biol 1992;18:205-12. [Crossref] [PubMed]
- Rouyer J, Varray F, Pozo E, Basset O, Cachard C, Lavarello R. Evaluation of a frequency-domain ultrasonic imaging attenuation compensation technique. Conf Proc IEEE Eng Med Biol Soc 2015;2015:1560-3. [PubMed]
- Ilyina N, Hermans J, Verboven E, Van Den Abeele K, D'Agostino E, D'hooge J. Attenuation estimation by repeatedly solving the forward scattering problem. Ultrasonics 2018;84:201-9. [Crossref] [PubMed]
- Unsgaard G, Gronningsaeter A, Ommedal S, Nagelhus Hernes TA. Brain operations guided by real-time two-dimensional ultrasound: new possibilities as a result of improved image quality. Neurosurgery 2002;51:402-11; discussion 411-2. [Crossref] [PubMed]
- Alomari A, Jaspers C, Reinbold WD, Feldkamp J, Knappe UJ. Use of intraoperative intracavitary (direct-contact) ultrasound for resection control in transsphenoidal surgery for pituitary tumors: evaluation of a microsurgical series. Acta Neurochir (Wien) 2019;161:109-17. [Crossref] [PubMed]
- Morin F, Courtecuisse H, Reinertsen I, Le Lann F, Palombi O, Payan Y, Chabanas M. Brain-shift compensation using intraoperative ultrasound and constraint-based biomechanical simulation. Med Image Anal 2017;40:133-53. [Crossref] [PubMed]
- Chen SJ, Reinertsen I, Coupé P, Yan CX, Mercier L, Del Maestro DR, Collins DL. Validation of a hybrid Doppler ultrasound vessel-based registration algorithm for neurosurgery. Int J Comput Assist Radiol Surg 2012;7:667-85. [Crossref] [PubMed]
- Akeret K, Bellut D, Huppertz HJ, Ramantani G, König K, Serra C, Regli L, Krayenbühl N. Ultrasonographic features of focal cortical dysplasia and their relevance for epilepsy surgery. Neurosurg Focus 2018;45:E5. [Crossref] [PubMed]
- Kang J, Song I, Kim H, Kim H, Lee S, Choi Y, Chang HJ, Sohn DK, Yoo H. Rapid tissue histology using multichannel confocal fluorescence microscopy with focus tracking. Quant Imaging Med Surg 2018;8:884-93. [Crossref] [PubMed]
- Romeike BF, Meyer T, Reichart R, Kalff R, Petersen I, Dietzek B, Popp J. Coherent anti-Stokes Raman scattering and two photon excited fluorescence for neurosurgery. Clin Neurol Neurosurg 2015;131:42-6. [Crossref] [PubMed]
- Hollon T, Stummer W, Orringer D, Suero Molina E. Surgical Adjuncts to Increase the Extent of Resection: Intraoperative MRI, Fluorescence, and Raman Histology. Neurosurg Clin N Am 2019;30:65-74. [Crossref] [PubMed]
- Ganau M, Bosco A, Palma A, Corvaglia S, Parisse P, Fruk L, Beltrami AP, Cesselli D, Casalis L, Scoles G. A DNA-based nano-immunoassay for the label-free detection of glial fibrillary acidic protein in multicell lysates. Nanomedicine 2015;11:293-300. [Crossref] [PubMed]
- Jermyn M, Mercier J, Aubertin K, Desroches J, Urmey K, Karamchandiani J, Marple E, Guiot MC, Leblond F, Petrecca K. Highly Accurate Detection of Cancer In Situ with Intraoperative, Label-Free, Multimodal Optical Spectroscopy. Cancer Res 2017;77:3942-50. [Crossref] [PubMed]
- Ganau L, Prisco L, Ligarotti GKI, Ambu R, Ganau M. Understanding the Pathological Basis of Neurological Diseases Through Diagnostic Platforms Based on Innovations in Biomedical Engineering: New Concepts and Theranostics Perspectives. Medicines (Basel) 2018.5. [PubMed]
- Gibbs SL. Near infrared fluorescence for image-guided surgery. Quant Imaging Med Surg 2012;2:177-87. [PubMed]
- Eschbacher J, Martirosyan NL, Nakaji P, Sanai N, Preul MC, Smith KA, Coons SW, Spetzler RF. In vivo intraoperative confocal microscopy for real-time histopathological imaging of brain tumors. J Neurosurg 2012;116:854-60. [Crossref] [PubMed]
- Ganau M, Paris M, Syrmos N, Ganau L, Ligarotti GKI, Moghaddamjou A, Prisco L, Ambu R, Chibbaro S. How Nanotechnology and Biomedical Engineering Are Supporting the Identification of Predictive Biomarkers in Neuro-Oncology. Medicines (Basel) 2018;5:E23. [Crossref] [PubMed]
- Shi J, Tang Y, Yao J. Advances in super-resolution photoacoustic imaging. Quant Imaging Med Surg 2018;8:724-32. [Crossref] [PubMed]
- Kröger S, Niehoff AC, Jeibmann A, Sperling M, Paulus W, Stummer W, Karst U. Complementary Molecular and Elemental Mass-Spectrometric Imaging of Human Brain Tumors Resected by Fluorescence-Guided Surgery. Anal Chem 2018;90:12253-60. [Crossref] [PubMed]
- Ohue S, Kumon Y, Nagato S, Kohno S, Harada H, Nakagawa K, Kikuchi K, Miki H, Ohnishi T. Evaluation of intraoperative brain shift using an ultrasound-linked navigation system for brain tumor surgery. Neurol Med Chir (Tokyo) 2010;50:291-300. [Crossref] [PubMed]
- Drouin S, Kochanowska A, Kersten-Oertel M, Gerard IJ, Zelmann R, De Nigris D, Bériault S, Arbel T, Sirhan D, Sadikot AF, Hall JA, Sinclair DS, Petrecca K, DelMaestro RF, Collins DL. IBIS: an OR ready open-source platform for image-guided neurosurgery. Int J Comput Assist Radiol Surg 2017;12:363-78. [Crossref] [PubMed]
- Kaneko OF, Willmann JK. Ultrasound for molecular imaging and therapy in cancer. Quant Imaging Med Surg 2012;2:87-97. [PubMed]
- Pysz MA, Guracar I, Tian L, Willmann JK. Fast microbubble dwell-time based ultrasonic molecular imaging approach for quantification and monitoring of angiogenesis in cancer. Quant Imaging Med Surg 2012;2:68-80. [PubMed]
- Ruan H, Mather ML, Morgan SP. Ultrasound modulated optical tomography contrast enhancement with non-linear oscillation of microbubbles. Quant Imaging Med Surg 2015;5:9-16. [PubMed]
- Wu DF, He W, Lin S, Han B, Zee CS. Using Real-Time Fusion Imaging Constructed from Contrast Enhancement Ultrasonography and Magnetic Resonance Imaging for High Grade Glioma in Neurosurgery. World Neurosurg 2019. Epub ahead of print. [Crossref] [PubMed]
- Ganau M. Tackling gliomas with nanoformulated antineoplastic drugs: suitability of hyaluronic acid nanoparticles. Clin Transl Oncol 2014;16:220-3. [Crossref] [PubMed]
- Ganau M, Syrmos NC, D'Arco F, Ganau L, Chibbaro S, Prisco L, Ligarotti GKI, Ambu R, Soddu A. Enhancing contrast agents and radiotracers performance through hyaluronic acid-coating in neuroradiology and nuclear medicine. Hell J Nucl Med 2017;20:166-8. [PubMed]
- Lee JH, Park G, Hong GH, Choi J, Choi HS. Design considerations for targeted optical contrast agents. Quant Imaging Med Surg 2012;2:266-73. [PubMed]
- Giussani C, Riva M, Djonov V, Beretta S, Prada F, Sganzerla E. Brain ultrasound rehearsal before surgery: A pilot cadaver study. Clin Anat 2017;30:1017-23. [Crossref] [PubMed]